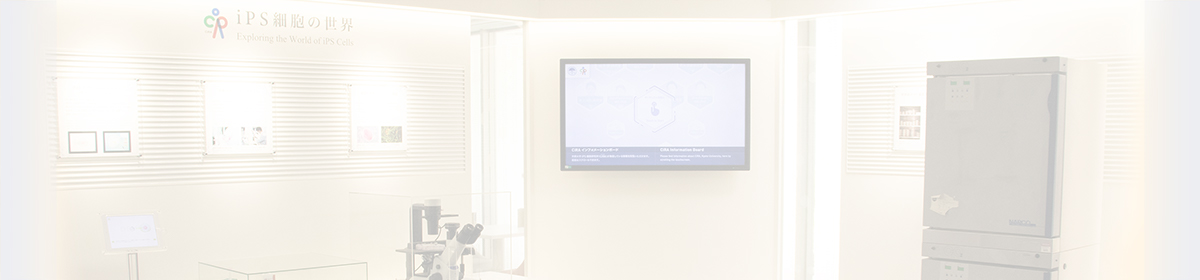
News & Events
News & Events
News
February 27, 2014
Current status of iPS cell technology and progress toward medical applications
by Shinya Yamanaka, M.D., Ph.D.
I was amazed by the recent reports on the STAP phenomenon (Obokata et al., 2014a, 2014b). We would like to contribute to the advancement of this new research, taking advantage of the expertise we have accumulated through the development of iPS cell technology. Since its first demonstration in 2006, iPS cell technology has made great advances through the numerous studies carried out by us and other scientists around the world. I would like to use this opportunity to give an update on the current status of iPS cell technology and progress toward medical applications.
1. Advancement in iPS cell technology to prevent tumor formation
When we first announced the generation of mouse iPS cells in 2006, the initial method utilized the oncogene c-Myc as one of the reprogramming factors. We also used retroviral vectors, which could cause tumors when integrated into the genome of host somatic cells. However, we no longer use either c-Myc or retroviral vectors to generate iPS cells for regenerative medicine. We have replaced c-Myc with safer factors and retroviruses with plasmids, which are not integrated into the host genome.
The safety of iPSCs generated with the current method has been undergoing tests in preclinical studies using experimental animals. As a result, Japan's Ministry of Health, Labour and Welfare last year approved a clinical study in which autologous iPS cell-derived retinal pigment epithelium cells were to be transplanted into a small number of patients with age-related macular degeneration. A team led by Dr. Masayo Takahashi of the RIKEN Center for Developmental Biology is now progressing with the study.
The safety of STAP stem cells should be exhaustively examined once they have been generated from human cells.
The efficiency of iPS cell generation has been significantly improved since its first demonstration. In the initial report of 2006, 0.1 percent or less of fibroblasts became iPS cells after the introduction of reprogramming factors. In 2009, we demonstrated that efficiency could be increased up to 20 percent (Hong et al., 2009). In 2013, an Israeli team reported that nearly 100 percent of human and mouse cells can become iPS cells (Rais et al., 2013).
For future medical applications, STAP cells and STAP stem cells should be clearly distinguished. Embryonic stem (ES) cells and iPS cells have the ability to differentiate into any cell type in the body (pluripotency) and the ability to self-renew (proliferation). STAP cells meanwhile are pluripotent but not proliferative, but by culturing in special medium, they can be converted into STAP stem cells, which do self-renew and are pluripotent. The comparison with ES cells and iPS cells should therefore be made using not STAP cells, but STAP stem cells. According to the report by Obokata et al., 20 percent of neonate mouse lymphocytes exposed to acidic stress survived, and one-third to one-half of the surviving cells became STAP cells, which indicates that the induction efficiency of STAP cells is less than 10 percent. The paper also indicates that successful conversion from STAP cells into STAP stem cells takes place once or twice in every ten cases. The efficiency of STAP stem cell generation from adult mice and humans has yet to be determined.
However, these efficiency figures are not very important from a practical point of view, since more than 100 iPS cell colonies can be obtained from 1 million somatic cells even at an efficiency rate of 0.1%. Reproducibility and compatibility are far more important, as explained below.
The reproducibility of iPS cell technology is extremely high. Hundreds of laboratories around the world are using the technology. Human iPS cells can be generated from virtually all donor individuals, regardless of age or health condition.
Another advantage of iPS cell technology is its compatibility with ES cells, which were first announced in mice in 1981 (Evans and Kaufman, 1981) and in humans in 1998 (Thomson et al., 1998). Techniques developed for ES cell research, such as the culture medium and protocols for in vitro directed differentiation, can be applied to iPS cells. This compatibility enabled researchers who had worked on ES cells to move quickly to iPS cells soon after the generation of human iPS cells was reported in 2007 (Takahashi et al., 2007).
There have been other stem cell technologies that made headlines when they were announced (e.g. MAPCs; Jiang et al., 2002). But due to their lack of reproducibility and compatibility, the technologies have not been taken up by many other researchers.
For STAP stem cells to be widely adopted, it is likewise important to first confirm their reproducibility and compatibility with ES cells and iPS cells. Otherwise, great effort and cost will be required to develop from scratch the protocols necessary for clinical research and clinical trials. It is also important to secure patents to disseminate the new technology.
Human iPS cell technology is highly reproducible and has good compatibility with human ES cells. We are now in the final phase of confirming its safety and efficacy through clinical research. STAP stem cell technology will be examined for reproducibility, compatibility and safety once cell lines have been established from human cells.
STAP cells are a major discovery in stem cell biology which will help us to elucidate the process of nuclear reprogramming and may lead in the future to new therapies, such as ways of repairing or regenerating organs within the body. CiRA wishes to contribute to STAP technology and to cooperate fully with RIKEN and other research institutes in exploring this emerging technology.
* Dr. Shinya Yamanaka is the director of the Center for iPS Cell Research and Application, Kyoto University and also serves as a senior investigator at the Gladstone Institute of Cardiovascular Disease in San Francisco.
Evans, M.J., and Kaufman, M.H. (1981). Establishment in culture of pluripotential cells from mouse embryos. Nature 292, 154-156.
Hong, H., Takahashi, K., Ichisaka, T., Aoi, T., Kanagawa, O., Nakagawa, M., Okita, K., and Yamanaka, S. (2009). Suppression of induced pluripotent stem cell generation by the p53-p21 pathway. Nature 460, 1132-1135.
Jiang, Y., Jahagirdar, B.N., Reinhardt, R.L., Schwartz, R.E., Keene, C.D., Ortiz-Gonzalez, X.R., Reyes, M., Lenvik, T., Lund, T., Blackstad, M., et al. (2002). Pluripotency of mesenchymal stem cells derived from adult marrow. Nature 418, 41-49.
Obokata, H., Sasai, Y., Niwa, H., Kadota, M., Andrabi, M., Takata, N., Tokoro, M., Terashita, Y., Yonemura, S., Vacanti, C.A., et al. (2014a). Bidirectional developmental potential in reprogrammed cells with acquired pluripotency. Nature 505, 676-680.
Obokata, H., Wakayama, T., Sasai, Y., Kojima, K., Vacanti, M.P., Niwa, H., Yamato, M., and Vacanti, C.A. (2014b). Stimulus-triggered fate conversion of somatic cells into pluripotency. Nature 505, 641-647.
Rais, Y., Zviran, A., Geula, S., Gafni, O., Chomsky, E., Viukov, S., Mansour, A.A., Caspi, I., Krupalnik, V., Zerbib, M., et al. (2013). Deterministic direct reprogramming of somatic cells to pluripotency. Nature 502, 65-70.
Takahashi, K., Tanabe, K., Ohnuki, M., Narita, M., Ichisaka, T., Tomoda, K., and Yamanaka, S. (2007). Induction of Pluripotent Stem Cells from Adult Human Fibroblasts by Defined Factors. Cell 131, 861-872.
Thomson, J.A., Itskovitz-Eldor, J., Shapiro, S.S., Waknitz, M.A., Swiergiel, J.J., Marshall, V.S., and Jones, J.M. (1998). Embryonic Stem Cell Lines Derived from Human Blastocysts. Science 282, 1145-1147.